Introduction
Recovery from training encompasses a multifaceted process involving various physiological systems within the human body [1]. It comprises a range of physiological and psychological mechanisms that enable the body to repair, adapt, and rejuvenate following physical activity. These processes include tissue repair, replenishment of glycogen stores, restoration of hormonal balance, reduction of inflammation, recovery of the central nervous system, and psychological restoration [2]. The duration and magnitude of the recovery process is contingent upon the specific stress and stimulus imposed by the exercise or training session [3].
Resistance training, characterized by movements performed against an external resistance, can exert substantial mechanical or metabolic strain, potentially resulting in muscle damage and fatigue [4–6]. The extent of fatigue primarily depends on training volume and intensity [7]. Training volume refers to the number of sets and repetitions performed in an exercise, while training intensity pertains to the load applied, typically expressed as a percentage of an individual’s one-repetition maximum in that specific exercise [8]. The relationship between training volume and intensity has been extensively studied, revealing an inverse association wherein higher training volume is generally accompanied by lower intensity, and a higher training intensity is generally accompanied by a lower training volume [9]. Greater training volumes are associated with heightened metabolic stress and muscle damage, which can impede force production capacity and the ability to train at high intensity [10]. High training volumes are commonly employed to optimize muscle endurance, and has been often associated with training programs to enhance muscle growth [8]. High volume resistance training programs are generally characterized by a high number of repetitions (≥10) at a moderate intensity (≤70% of one-repetition maximum) [11]. Conversely, higher training intensities involve loads nearing an individual’s maximal strength, thereby limiting the capacity to perform a high number of repetitions [9]. High-intensity training is frequently utilized for maximal strength development, power enhancement, and neuromuscular adaptations, typically involving a lower number of repetitions [4–6] per set at 85–90% of one-repetition maximum [11]. The high-intensity training paradigm also appears to have significant effects on subsequent neuromuscular performance. Doma and Deakin [12] reported greater declines in time-to-exhaustion running trials up to six hours post-workout following high-intensity resistance training compared to lower intensity resistance training programs. In addition to differences in training modalities, alteration in training intensity and volume also exhibit distinct recovery responses. Numerous studies have reported prolonged recovery time, greater strength loss, and increased inflammatory response following training programs of high volume compared to high-intensity training [13–16].
The recovery response between high volume and high intensity training has been extensively investigated. However, comparisons of these two training paradigms on cognitive function and reaction time is less understood. Previous research examined the effect of high volume and high intensity training on circulating brain-derived neurotrophic factor (BDNF), a neurotrophic factor implicated in neuronal development and cognitive function [17]. Contrary to the authors’ hypothesis, no differences in the BDNF response were observed between high-intensity (90% one-repetition maximum) and high-volume (70% one-repetition maximum) resistance training programs. Although it is well-established that exercise enhances cognitive function in the long term [18], the impact of acute exercise on cognitive function has yielded contrasting findings. One study demonstrated that high-intensity aerobic exercise (80% heart rate reserve) resulted in impaired cognitive performance immediately following and 20-minutes post-exercise compared to lower intensity aerobic exercise [19]. Consistent with these findings, an additional study reported impaired executive control in subjects who engaged in 30-minutes of endurance exercise at the ventilatory threshold compared to those who performed the same exercise at 75% of the ventilatory threshold [20]. However, recent studies have suggested that moderate intensity endurance exercise (between 64%-76% of maximal aerobic capacity) [21] and high intensity interval training [22] can improve acute cognitive function. A recent study by chen and colleagues [23] have reported significant acute improvements in executive functioning immediately following combined endurance and resistance training. Improved cognitive function seen following acute exercise is thought to be associated with significant elevations in dopamine concentrations that can enhance reaction time [24].
The focus of most studies that have examined the recovery response from exercise and its effect on acute cognitive function has primarily focused on endurance performance. To the best of our knowledge, there does not appear to be any previous investigations that have examined the effect of resistance training on acute cognitive performance, nor has there been any research comparing the effect of different resistance training paradigms on changes in acute cognitive function. Therefore, the purpose of this study was to compare a high volume (HV) and high intensity (HI) resistance training exercise program on changes in cognitive performance. In addition, a secondary purpose of the study was to determine whether acute changes in physical and cognitive performance follow similar patterns of fatigue and recovery. Our initial hypothesis is that high intensity exercise will have greater effects on neuro-muscular performance changes than low intensity exercise. Secondly, we hypothesize that physical and cognitive recovery measures will differ between low and high intensity resistance exercise. Specifically, that greater declines will be seen in high intensity exercise compared to the lower intensity exercise paradigm.
Material and methods
Participants
Eight recreationally trained men (age = 27.8 ± 1.6 years, body mass = 85.5 ± 11.2 kg, height = 178.4 ± 8.3 cm) with at least one-year of resistance training experience (6.4 ± 3.8 y), including specific experience performing the squat exercise, volunteered to participate in this study.
Study design
The investigation was performed as a randomized, crossover design as part of the experimental protocol. The participants visited the Human Performance Laboratory (HPL) on three separate occasions. During the initial visit, participants underwent assessments for one-repetition maximal strength (1-rM) in the back squat exercise. One week after the initial visit, participants returned to the laboratory and were randomly assigned to either the HV or HI exercise protocol, using a simple randomization technique. The participants reported to the HPL for the third and final visit one week following the previous visit and proceeded to perform the alternative exercise program both exercise protocols required the participant to perform six sets of the squat exercise. The HV exercise protocol required the participant to perform 15-20 repetitions per set at 60% of their previously measured 1-rM in the barbell back squat exercise. During the HI exercise protocol participants performed 3-5 repetitions per set at 90% of their previously measured 1-rM. The recovery period between sets for the HV and HI exercise protocols was set at 1 min and 3 min, respectively. If participants were unable to complete the prescribed number of repetitions per set during either trial, the load for the subsequent set was reduced by 5 kg to ensure they could achieve the required repetitions. All exercise sessions occurred at the same time of day for both training protocols.
Force, reaction, and cognitive function measures were conducted at baseline (BL), immediately post-exercise (IP) and at 30- (30P) and 60-minutes (60P) post-exercise. During each testing period participants performed a maximal effort isometric mid-thigh pull (IMTP) on a force plate (400S+ Performance Plate, Fitness Technology, Adelaide, Australia). Peak force and the rate of force development (RFD) were subsequently calculated. Reactive agility was measured using the Blazepod (Play Coyotta Ltd, Tel Aviv, Israel) reactive agility device, and cognitive function was measured using step 3 of the Sport concussion Assessment Tool (SCAT5).
Physical performance measures
Before conducting the 1-RM barbell squat test, participants performed a standardized warm-up [25]. The warm-up consisted of performing 5 min on a cycle ergometer, 10 body weight squats, 10 body weight walking lunges, 10 dynamic walking hamstring stretches, and 10 dynamic walking. The 1-RM test protocol for the barbell back squat was performed as previously described [25]. Briefly, participants performed two warm-up sets with a resistance of approximately 40– 60% and 60–80% of their perceived 1-RM, respectively. The third set was the participant’s first attempt for their 1-RM. If the set was successfully completed, then weight was added, and another set was attempted. If the set was not successfully completed, then the weight was reduced, and another set was attempted. A 3–5-min rest period was provided between each set. The process of adding and removing weight continued until a 1-RM was reached. Trials not meeting the range of motion criteria were discarded. The range of motion criteria for the barbell back squat was reaching an angle of 90° between the hip and the knee joints. The range of motion was confirmed visually by one of the researchers. The 1-RM squat, range of motion criteria and all resistance training sessions were supervised by an experienced, certified strength and conditioning coach.
Maximal isometric strength was measured using an isometric mid-thigh pull (IMTP) test using a power rack that permitted fixation of the bar at a height that corresponded to the participant’s mid-thigh while standing on the force plate. Force-time data were captured with a sampling frequency of 1,000 Hz using the BMS version 2 (BMSv2) software program. Participants were instructed to position their feet directly beneath the centre of the bar, approximately hip width apart and hold the bar using a “clean” grip. At the beginning of each trial, participants were instructed to “pull as hard as possible” and to “keep pulling until you receive the signal from the investigator to stop” [26]. The stop signal was given when the force output displayed either plateaued for 1 s or declined. Each subject performed two trials and a 2-minute rest between attempts. Data from the force plate regarding peak force and RFD were recorded from the trial with the highest peak force output. Rate of force development was measured at specific time points of 0–150, 0–200, and 0–250 ms.
Reactive agility assessment
To measure reactive agility, participants were required to perform both the side shuffle and the 3-m reactive agility drills as previously described [27].
Side shuffle reactive drill
Five blazepod devices were arranged in a parallel configuration, spaced 1 meter apart from each other. The participants positioned themselves directly in front of the middle pod. A computer-generated countdown signaled the initiation of the drill. Following the “go” command, the participant began to move in place until one of the pods illuminated. The participant then laterally shuffled to the lit pod and tap it with his closest hand. He would then move back in place and begin to laterally shuffle to the opposite side or until the next pod would illuminate. He continued to react to each pod illumination for the 20-second trial. The pods illuminated in a random sequence. The average reaction time calculated from the moment that the pod illuminated until the participant tapped the light was recorded. Each participant had a single opportunity to perform the test.
3-m reactive agility drill
The execution of this drill was performed in an identical manner as the side shuffle reactive drill, with the exception that the participant initiated the drill from a starting position three meters in front of the middle cone and returned to that same position after responding to each illuminated pod. Similar to the previous drill, this activity lasted for a duration of 20 s, and the average reaction time was recorded.
Cognitive function assessment
Cognitive function was assessed using only step 3 of the Sport concussion Assessment Tool, fifth Edition (ScAT5) [28]. The ScAT5 is considered to be a valid and reliable sideline assessment tool to assess changes in cognitive function and risk for concussion [29]. Step 3 of the SCAT focuses on the cognitive function portion of the assessment, specifically examining orientation, acute memory, concentration, and delayed memory. Questions regarding orientation focused on the participant answering questions to where they are at this precise moment regarding year, month, day of the week, date, and time. Acute memory required the test administrator to read a list of 10 words (one word per second) and ask the participant to repeat as many words as possible. This was repeated three times using the same list of words. The third trial was timed. During each testing session, a different list of words was used. After the evaluation of acute memory, participants underwent an assessment for concentration. This test required the test administrator to read a string of numbers ranging from three to six digits. Following each number sequence, the participants were instructed to repeat the numbers in reverse order. A different number sequence was utilized for each testing session. The participants were then asked to recite the months of the year in reverse order. The final assessment focused on delayed memory and took place five minutes following the immediate memory evaluation and required the participant to recall as many of the 10 words as they could remember. The SCAT5 was conducted in the participants native language (e.g., Hebrew) and the same administrator conducted all cognitive assessment tests. To the best of our knowledge, this is the first study to use the SCAT5 to examine cognitive function following resistance exercise.
Statistical analyses
A Shapiro–Wilk test was conducted for testing normality in maximal strength, RFD, reactive agility, and cognitive function. If the assumption of sphericity was violated, a Greenhouse-Geisser correction was applied. Strength performance, reactive agility, and cognitive function were analysed using a two-factor (trial × time) analysis of variance (ANOVA) with repeated measures. In the event of a significant F ratio, a Tukey post hoc analysis was performed to examine between group differences. For parameters that did not follow a normal distribution we performed the non-parametric Friedman test with Dunn’s multiple comparisons [30]. Significance was accepted at an alpha level of p 0.05, and all data are reported as mean ± SD. All statistical analyses were performed using GraphPad Prism (version 8.4.2). The required sample size based upon previous research comparing high intensity versus high volume training [12] using a repeated measures two-factor ANOVA (effect size 0.437, error = 0.05 and power = 0.80) was more than 8 participants using G*power 3.1.9.4.
To complement our null hypothesis testing and to make inferences about the true effects of the two different exercise protocols, data were further analysed using magnitude-based inferences (MBI) [31]. This statistical analysis procedure is often used in sport science research when sample sizes are often small. In addition, it puts greater emphasis on the effect size of the sample. Several studies have suggested that the MBI analysis is an effective statistical tool for null hypothesis testing to reduce interpretation errors. Data were calculated from 90% CI and analysed as previously described [32]. Differences in the change of the △ scores between ACC vs PL at all time points were analysed using the p-value from independent t-tests to determine a mechanistic inference utilizing a published spreadsheet [33]. All data are expressed as a mean effect ± SD, with percent chances of a beneficial, trivial, or negative outcome. Qualitative inferences, based on quantitative chances, were assessed as: < 1% almost certainly not, 1–5% very unlikely, 5–25% unlikely, 25–75% possibly, 75–95% likely, 95–99% very likely, and > 99% almost certainly [34]. If there was a greater than 5% chance that the true value was either greater or lesser, indicating that the CI was overlapping multiple thresholds, the effect was considered to be mechanistically unclear [34]. The smallest non-trivial change, or smallest worthwhile change, was set at 20% of the grand standard deviation for all PRE-values [34].
Ethical approval
The research related to human use has complied with all the relevant national regulations and institutional policies, has followed the tenets of the Declaration of Helsinki, and has been approved by the Institutional review board of Ariel University (approval No.: AU-HEA-JH-20230214).
Informed consent
Following an explanation of all procedures, including the risks and benefits associated with volunteering for the study, each participant provided his informed consent.
Results
The 1-rM for the squat exercise of the participants was 132.2 ± 10.0 kg. The volume of training was significantly different (p < 0.0001) between HV (7502 ± 988.6 kg) and HI (2310 ± 293.4 kg) exercise protocols.
Maximal isometric force and RFD
Results of the IMTP can be observed in Figures 1a-d. When examining the differences in recovery between the HV and HI training protocols on isometric peak force, no significant group x time interaction (F = 0.954; p = 424) was noted, but a significant time effect (F = 4.195; p = 0.011, Figure 1a) was found. Post-hoc analysis for time revealed a significant decrease in peak force only at 60P compared to bL (p = 0.008). As a result of a violation in normality in the RFD at 150ms, we used the non-parametric Friedman test to examine differences between the groups over time [25]. No significant change from bL was noted during the one-hour recovery period following the HV or HI training protocols (see Figure 1b) (Friedman statistic = 3.75, p = 0.29, Friedman statistic = 4.65, p = 0.20, respectively). Examination of changes in RFD at 200 ms and 250 ms (see Figures 1c and 1d) between the groups resulted in no significant group x time interactions (F = 0.300; p = 0.827 and F = 0.338; p = 0.797, respectively). However, significant time effects (F = 5.707; p = 0.002, F = 5.717; p = 0.002) were found for the RFD at 200 ms and 250 ms, respectively. Post-hoc analysis for time revealed a significant decrease from BL at both 30P and 60P (p = 0.011; p = 0.002, p = 0.029; p = 0.001, respectively). MBI analysis (see Table 1) revealed a possibly greater reduction (63.05%) in peak force at 30P following the HI session compared to the HV protocol. Analysis of RFD at 150 ms, 200 ms, and 250 ms indicated that both training protocols were unclear for reducing RFD at IP, 30P, and 60P.
Figure 1
Changes in isometric peak force and RFD at 150 ms, 200 ms, and 250. A – changes in isometric peak force, B – changes in RFD at 250 ms, C – changes in RFD at 200 ms, D – changes in RFD at 150 ms
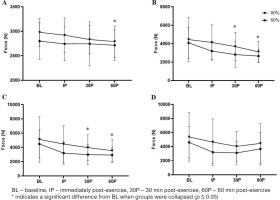
Table 1
Magnitude-based inferences on change scores in performance measurements
Reactive agility
All changes in reactive agility can be observed in Table 2a. No significant group × time interaction (F = 0.276; p = 0.842) or significant time effect (F = 0.546; p = 0.653) for the side-shuffle reactive agility test were noted. Similarly, no significant group × time interaction (F = 1.649; p = 0.193) was observed for the 3-m reactive agility test. However, a significant time effect was seen (F = 2.950; p = 0.043). Post-hoc analysis revealed a significant increase in 3-m reaction time at IP (p = 0.034). Performance returned to bL levels by 30P. MbI analysis (see Table 2b) indicated no clear differences between HV or HI resistance exercise protocols for changes in reaction time in the side-shuffle reactive agility test. However, MbI analysis for the 3-m reactive agility drill indicated a likely negative effect (90.20%) on 3-m reactive agility at IP following HV compared to the HI training protocol.
Table 2a
Reactive agility
Table 2b
Magnitude-based inferences on change scores in reactive agility
Cognitive function
The effect of HV and HI resistance exercise protocols on changes in cognitive function can be observed in Table 3a. No significant group x time interaction or significant time effect (F = 0.411; p = 0.746 and F = 2.10; p = 0.114) were observed for changes in acute memory between HV and HI, respectively. As a result of a violation in normality, we used a nonparametric Friedman test to analyse concentration and delayed memory. No significant differences were noted in concertation across all time points in either the HV or HI protocols (Friedman statistic = 4.846; p = 0.183, Friedman statistic = 3.600; p = 0.308, respectively). However, when analysing delayed memory, an independent analysis for each training intervention indicated a significant main effect for time only in the HV compared to HI training intervention (Friedman statistic = 11.74; p = 0.008, Friedman statistic = 2.526; p = 0.47, respectively). Dunn’s multiple comparison test revealed a significant decrease in delayed memory from BL to both IP and 30P (p = 0.035, p = 0.046, respectfully). MBI analysis (see Table 3b) results in no clear differences between HV and HI for acute memory, concentration, or delayed memory at any time point.
Table 3a
Cognitive function
Table 3b
Magnitude-based inferences on change scores in cognitive function
Discussion
This study compared fatigue patterns in force capability, reactive agility, and cognitive function from two popular resistance training paradigms. Results indicated no significant differences between the two training paradigms in any of the physical and cognitive performance measures. However, the pattern of change did appear to differ. Fatigue resulting in significant declines in physical function (e.g., force capability) appeared at both 30- and 60- min post-exercise in both HV and HI. However, changes in 3-m reactive agility revealed a significant decline immediately following completion of both training protocols but returned to baseline levels by 30-min post-exercise. Changes in cognitive function indicated a significant decrease in the delayed memory task at IP and 30 min following the HV training protocol, but no changes were observed in delayed memory following the HI training protocol. The use of a complementary analysis using MBI provided further clarity on these changes. It appeared that the HI training protocol resulted in greater perturbations in force compared to HV at 30P, while the HV training protocol resulted in greater perturbations in reactive agility compared to HI at IP. MBI analysis indicated that differences between HV and HI in any cognitive measure was unclear.
Recovery from physical activity encompasses a complex process that engages multiple physiological systems within the human body. However, an athlete is generally accepted as recovered when their performance capabilities return to baseline levels following a bout of exercise [30]. Various forms of exercise impose distinct stressors on the body, possibly resulting in differences in recovery that is likely related to differences in the metabolic and mechanical stress of each training paradigm [6]. HI intensity resistance training has been shown to result in significantly greater elevations in muscle damage, as reflected by acute elevations in muscle damage biomarkers myoglobin and lactate dehydrogenase [35]. These changes were suggested to be related to the greater motor unit recruitment in the high-intensity resistance training paradigm [35]. Interestingly, the higher volume training was associated with a greater sum of motor unit activation. Over chronic training, the greater cellular damage associated with the high intensity training is thought to contribute to greater strength gains compared to HV training paradigms [36, 37].
HV resistance exercise predominantly taxes the glycolytic energy system, resulting in elevated levels of acidosis and metabolites based on the metabolic demands of the high volume of training [11]. Previous research has reported that the accumulation of these metabolites leads to increased muscle soreness and declines in strength [16, 38]. Bartolomei et al. compared the recovery responses following HV or HI exercise protocols [13]. Their findings demonstrated a significant decrease in countermovement jump power production and peak torque after 30 minutes following the HV resistance training protocol compared to the HI protocol. Furthermore, an elevation in inflammatory markers at the 30-minute post-exercise was observed only following the HV protocol. In contrast, our results revealed no significant differences in performance between the two experimental protocols. However, the significant time effects for peak force and RFD at 200 and 250 ms observed at 30P and 60P do suggest that changes in muscle force ability, regardless of training paradigm, occur at a different time frame than changes in cognitive function and reactive agility.
MBI analysis revealed a possible greater decrease in peak force at 30P for HI compared to HV. The possible attenuation in peak force following the HI protocol may be related to the high load (90% of 1RM) that was used in the HI training protocol, a training paradigm acknowledged for its preferential recruitment of the larger type II motor units [39]. It is possible that the HI protocol’s application of high loads resulted in a transient exhaustion of the type II muscle fibres, which would have been primarily and preferentially recruited during the IMTP assessment. It is possible that lower threshold motor units were primarily recruited during the HV training program and the higher threshold units, which were likely recruited during the IMTP, were not as fatigued contributing to the observed results. Regardless, this level of fatigue appeared to be resolved by 60P.
Agility is often defined as the ability to change direction as quickly as possible in response to a stimulus [25]. Agility can be further defined as the ability to react to a stimulus, whether it be the flashing of a light, movement of a hand or an opponent. This may be referred to as reactive agility because the athlete reacts to an external stimulus [27]. Exercise-induced fatigue can detrimentally impact agility performance and reaction time, reduce explosive power and coordination, and disrupt neuromuscular communication [40–42]. To the best of our knowledge, no study to date has compared HV and HI resistance training programs on reactive agility. While we did not find any significant differences between the two protocols, MBI analysis revealed a likely greater reduction in reactive agility performance at IP following the HV protocol compared to the HI protocol. Although speculative, this decrement is likely related to the high acidosis and muscle soreness associated with HV resistance training compared to HI resistance training [6, 13, 43]. In addition, high volume resistance training, or training to failure, is also associated with a greater decrease in biomechanical properties and muscle damage compared to resistance training performed not to failure [44]. regardless, reactive agility returned to BL levels by 30P, suggesting that changes in performance were related more to an acute metabolic stress and not muscle damage, that once resolved allowed performance levels to return to BL.
Cognitive function encompasses diverse mental processes such as memory, reasoning, attention, problem-solving, inhibitory control, and flexible thinking [45]. The present study reported a significant reduction in cognitive function, specifically in delayed memory, only after the HV training protocol at IP and 30P. This specific drop in cognitive function may be explained by the hypofrontality theory, which suggests that as physical activity intensifies, the prefrontal cortex exhibits reduced activity, leading to a state of “hypofrontality” [46]. The decrease in neural activity in the prefrontal cortex is believed to result in a temporary impairment of executive functions [43]. During intense exercise, there is an increased demand for oxygen and glucose by the muscles to support their activity. This can lead to a decrease in the availability of these resources to the prefrontal cortex, impairing its function [47, 48]. Our findings appear to support this, as evidenced by the decline in delayed memory following the HV training intervention. The higher metabolic stress associated with the HV resistance training protocol may have impeded executive function within the prefrontal cortex, which appears to have recovered by 60P. In addition, the higher volume of training may have resulted in a greater shunting of blood to the exercising musculature. Despite blood to vital organs generally being maintained during exercise, there is still a reduction in total blood volume within the brain [19]. Although we did not measure changes in blood volume, it is possible that greater blood shunting occurred during the HV protocol that contributed to acute changes in delayed memory, which returned to BL levels as blood volume within the brain returned to its normal concentrations. It is worth noting that while the hypofrontality theory has been studied in the context of endurance exercise, its relevance to resistance exercise warrants further investigation.
There are a number of limitations to this investigation. Although this was a randomized cross-over design study, the relatively low number of participants may have limited our results. The use of the MbI was primarily done to compensate for the low number of participants and add further understanding to the study. In addition, it should be acknowledged that although the participants were experienced, they were still recreational athletes. It would not be appropriate to extrapolate the results of this study to a competitive group of athletes. Participants were all men, and whether these results would be similar in women remains to be evaluated. In addition, much of our discussion was speculative as limited physiological measures were examined. The results of this study do provide good impetus to further examine this question using more in-depth physiological measures, such as biochemical and hormonal changes. Interestingly, the data from this study does suggest that changes in peak force and the rate of force development appear to occur later in the recovery cycle compared to changes in cognitive function or reactive agility, likely related to the immediate fatigue related to the metabolic stress of the HV program. In conclusion, results of this study indicate that participants engaging in HV resistance training are more susceptible to experiencing performance declines in reaction time and cognitive function than HI training. This has important implications for coaches and trainers to consider providing different recovery times for individuals performing these different training paradigms. This is especially relevant in periodized training programs in which coaches need to be cognizant of the potential effects changes in acute cognitive performance and reaction time as the training paradigm changes.