Introduction
Badminton boasts immense popularity worldwide, with an estimated 200 million participants, making it the second most popular sport globally, following football [1]. In Asia, badminton surpasses even football in terms of popularity [2].
Mastering the smash is an essential skill in badminton, as it serves as the primary scoring technique for athletes [3], especially in doubles matches, where successful smashes can not only directly translate to points but also boost team morale [4]. However, as player training intensifies and defensive capabilities improve, single smashes become less effective. To overcome this challenge, athletes employ smashes from various distances to exploit the opponent’s weaknesses and score directly or indirectly [4].
Precisely controlling the landing point of a smash during badminton gameplay remains a significant challenge for athletes. The fast pace inherent to competitive matches further complicates this task, as adjustments mid-swing often lead to increased stroke errors. Existing research on smash accuracy primarily focuses on the upper extremity segments, including the shoulder, elbow, and wrist [5, 6]. This emphasis aligns with previous studies demonstrating that upper limb muscle activity exhibits the most significant contribution during the smash compared to other body regions [7]. Accordingly, experiments investigating upper limb mechanics across various smashing distances are warranted to gain a deeper understanding of the mechanics governing effective smashing, particularly regarding improved landing point control.
In recent years, several studies have shed light on the biomechanics of the smash in badminton. Wrist muscle activation is now understood to be crucial for accuracy, as evidenced by research showing a positive correlation between smashing accuracy and wrist muscle power [7]. Additionally, shoulder joint internal rotation acceleration is a significant factor influencing the speed of badminton [5]. Furthermore, studies comparing badminton techniques such as high balls, drop shots, and smashes consistently highlight the importance of the racket’s head speed, which is significantly correlated with the angular velocity of the upper limb joints [8, 9]. Similarly, research by Salim et al. [3] demonstrated a strong correlation between shoulder internal rotation velocity and elbow extension angular velocity with smashing speed. Examining muscle activation patterns through EMG analysis, Tsai et al. [10] found significant differences in wrist joint musculature between smashes and drop shots. Sakurai and Oht- suki’s [7] work on upper limb muscles concerning accuracy revealed a significant role for distal muscles (wrist flexors and extensors). Additionally, the biceps displayed activation during the preparatory phase, while the triceps played a crucial role at contact [7]. Finally, a cross-sectional study identified a significant correlation between upper extremity explosive power and speed of acquisition [11].
Prior research has established a clear connection between upper limb mechanics and smash effectiveness in badminton [6]. While existing studies have explored various aspects of smashing, a critical gap remains in our understanding of optimally controlling upper limb movements for efficient and accurate smashes across different distances. Furthermore, research on the biomechanics of smashing at varying distances is limited. Relying solely on repetitive practice to improve control at different distances can lead to overuse injuries [12]. Therefore, this study aims to investigate the impact of varying smash distances on arm motions and muscle activation patterns. We hypothesize that increased smash distance will result in changes in upper limb muscle activation, joint angles, and angular velocities. By analyzing these biomechanical factors during smashes performed from different distances, we hope to provide data-driven training recommendations for athletes, ultimately reducing errors and the risk of injuries associated with prolonged, uncontrolled practice. Additionally, this research can equip coaches with scientifically sound training methods to optimize athletes’ technical performance, enhance their competitive edge, and maximize their full potential.
Material and methods
Subjects
Ten collegiate athletes with a competitive badminton background were recruited for this study (mean age: 21 ± 1 years, weight: 71.9 ± 9.1 kg, height: 1.78 ± 2.24 m). All participants met the following inclusion criteria: (1) no history of surgery or injuries to the trunk or upper extremities, (2) no pain or discomfort in the upper limbs prior to testing, and (3) participation in badminton training four or more times per week. The participants were recruited from a local university and had a history of competing in national-level badminton championships.
Equipment and materials
Twelve infrared cameras (OptiTrack, Natural Point, Inc., Corvallis, OR, USA) operating at 120Hz captured kinematic data. A total of 61 reflective markers (57 on the body and 4 on the racket) were attached to the participant’s skin alongside six EMG sensors (Trigon, Avan- ti Sensor, Delays, USA). The software (OptiTrack, Natural Point, Inc., corvallis, OR, USA) synchronized the kinematic data (collected at 120Hz) with the EMG data (collected at 1200Hz). The EMG sensors were placed on the following muscles: anterior deltoid (DELTA), medial deltoid (DELTL), triceps brachii (TRIL), biceps brachii (BICLO), extensor carpi radialis (ECR), and flexor carpi ulnaris (FCU). All EMG reference standards adhered to the guidelines established by Bar- bero et al. [13]. The sensors were secured with tape to minimize external influences [14]. Prior to data collection, all participants underwent a series of maximum voluntary isometric contractions (MVCs) to determine their baseline muscle strength. Each MVC involved exerting a maximal force for five seconds in a specific posture, mimicking the target muscle action during a smash. For the anterior and medial deltoid, participants sat with their dominant arm hanging freely at their side, elbow flexed at 90 degrees, and shoulder slightly abducted. They then pushed maximally forward and backward within the plane parallel to their torso. To assess the biceps and triceps, subjects sat with their upper arm secured and wrists facing forward. Starting with a 90-degree elbow bend, they performed maximal elbow flexion and extension for five seconds each, with the tester stabilizing their forearm. Finally, wrist flexion and extension MVcs were obtained by having participants position their forearms on the stand with their hands extended outward, palms facing in- ward, and in a fist. They then exerted maximal force in flexion and extension directions for five seconds each, as described in the literature [13]. An automated service machine (SPT6000, SPTLOOKER, Guangzhou, china) was subsequently used to deliver shuttlecocks to designated areas during the experiment.
Experimental procedure
Each subject would first warm up for 10 minutes before the experiment, after which the researcher would reposition the tee according to the subject’s hitting habits to ensure that the badminton balls landed in roughly the same location and that the subject was able to hit the ball in a way that was comfortable for the subjects. By reading the previous studies [15, 16] and observing the motion model in motive, we divided the swing into four phases. We attached reflective markers to the badminton ball to ensure that it could be captured by the camera during its flight and at the moment of striking the ball by looking at it in motive (Figure 1). After all subjects confirmed their test movements, the formal experiment began. Participants were provided explicit instructions to position themselves within a specified area and engage in striking a shuttlecock propelled from a serving apparatus strategically situated on the opposing court. Employing a stable snap motion, subjects executed shuttlecock hits at different distances [17, 18]. The laboratory supplied both the test balls and rackets to ensure uniformity and to minimize potential disruptions from extraneous variables. Detailed depiction of the court configuration is presented in Figure 2.
Figure 1
There are four stages of the badminton swing: winding up, cocking, acceleration, and deceleration [15]. This paper studies and discusses the acceleration stage, also referred to as the contact stage
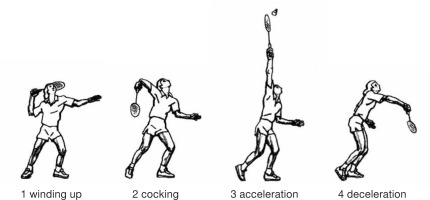
Figure 2
The ball was sent to a square area near the left bottom corner of 50 cm x 50 cm, 80 cm away from the left bottom line and 30 cm away from the left sideline. The subject stood in a square area 80 cm x 80 cm behind the landing point. The ball needed to be hit into three connected rectangular areas of 100 cm x 100 cm and labelled 1/2/3, respectively. To make players fixed hitting positions, automatic service machines were used when serving
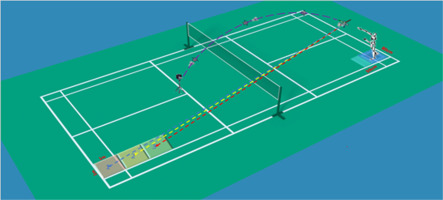
Analysis and processing
EMG data was sampled at 1200 Hz and subsequently filtered using a 10-400 Hz bandpass filter. The root mean square (RMS) with a 10 ms window was applied to smooth the EMG signals [19]. The average RMS amplitudes were calculated for the three trials and normalized by the maximum MVC values. Kinematic data processing involved the calculation of joint angles and angular velocities using Visual3D software. Positive and negative values were determined using a trunk-centred coordinate system (Y - forward/backward, X - left/right, Z - up/down). Trunk flexion/extension was defined as positive/negative, respectively, and adduction/abduction followed the same convention. Similarly, the inner/outer racket spin was assigned positive/negative values. Synchronization between kinematic and EMG data was achieved by visually identifying specific moments based on changes in joint angles and muscle activity patterns. The badminton forehand swing was segmented into four distinct stages (preparation, lead-in, impact, and follow-through) based on characteristic movement patterns [20]. Considering the relatively standardized swing patterns and joint trajectories observed across participants, the moment of impact was defined as the instant when the wrist joint reached the zero point on the X-axis. This study focused solely on the point of racket-shuttlecock contact at varying distances. Joint parameters (X and Z-axis) at impact were calculated using Visual3D. Subsequently, one-way analysis of variance (ANOVA) was conducted using SPSS 23.0 to assess the influence of distances on muscle activation (shoulder, elbow, and wrist), joint angular velocities, and joint angles at impact. The significance level was set at a p-value < 0.05.
Results
As illustrated in Figure 3, all investigated muscles exhibited low to moderate activity levels (3.1%-59.9% EMGMAX) during smashes performed at varying distances. Notably, the BICLO, TRIL, DELTA, DELTL, ECR, and FCU displayed their highest activation levels (5.2-59.9% EMGMAX) when smashing from the farthest distance. The TRIL, ECR, and FCU specifically demonstrated the ability to achieve moderate activity levels (30.4%-59.9% EMGMAX) across all distances, with particularly pronounced activity observed during far smashes. The results revealed a gradual increase in activation levels for the BICLO, TRIL, DELTA, DELTL, ECR, and FCU with increasing smash distances. Conversely, the BICLO, DELTL, and DELTA exhibited lower activity levels across all distances at impact (3.1%-33.7% EMGMAX). Interestingly, three muscles (not specified) displayed moderate activity (40.6%-59.9% EMGMAX), while the FCU reached its peak activity level (59.9% EMGMAX) at the moment of impact. Notably, the biceps displayed minimal activity throughout all three smash distances (3.1%- 5.2% EMGMAX). Statistical analyses revealed significant differences (p < 0.05) in the activation levels of the DELTA between close and far smashes, as well as between close and intermediate distances. Additionally, significant differences were observed between the ECR and FCU during close and far smashes (p < 0.005), with the ECR also exhibiting significant differences between close and intermediate distance smashes (p < 0.005). There were no significant differences detected regarding the activation levels of other muscles across the various smash distances.
Analysis of joint angles revealed no significant differences (F = 0.346, p > 0.05; F = 0.310, p > 0.05) in the shoulder joint (sagittal and horizontal planes) or elbow joint (sagittal plane) during badminton swings (table 1). However, a significant difference (F = 4.84, p < 0.05) was observed in the elbow joint angle on the horizontal plane. Post-hoc comparisons indicated that the internal rotation angle of the elbow joint decreased with increasing smash distances. Furthermore, multiple comparisons revealed significant differences (p < 0.001) between all three distances, with the most significant difference observed between close and far distances. Wrist joint angle analysis at impact revealed a significant difference on the sagittal plane (F = 10.88, p < 0.001). Post-hoc comparisons demonstrated that the wrist joint extension angle increased with increasing smash distances. Multiple comparisons indicated statistically significant differences in joint angles between close and intermediate distances, and between close and far distances (p < 0.001). However, no significant difference was observed between intermediate and far distances (p > 0.05). Wrist joint angle on the horizontal plane also exhibited a significant difference (F = 9.17, p < 0.001). Post-hoc comparisons revealed a decrease in the internal rotation angle of the wrist with increasing distances. All three distances showed statistically significant differences when using post-hoc tests (p < 0.005).
Table 1
Comparison of kinematic parameters of different upper limbs and the angle of the upper limb joints at the moment of the stroke
Table 2
Angular velocity of upper limb joints at the moment of the stroke
Analysis of upper limb angular velocities revealed significant differences (F = 26.3, p < 0.001) in the shoulder joint’s sagittal plane flexion across various smash distances. Post-hoc comparisons indicated a progressive increase in flexion angular velocity with increasing distances. All three distances displayed statistically significant differences (p < 0.001) from each other. Similarly, the shoulder joint’s horizontal plane internal rotation velocity exhibited significant differences between distances (F = 4.51, p < 0.05). Post-hoc analysis revealed a trend of increasing internal rotation velocity with increasing distances. However, a significant difference in internal rotation velocity was only observed between close and far smashes (p < 0.005) at impact.
The elbow joint’s sagittal plane flexion velocity also significantly varied across distances (F = 18.462, p < 0.001). Post-hoc comparisons confirmed that elbow flexion velocity increased with increasing distances, with all three distances exhibiting statistically significant differences in flexion velocity (p < 0.001) from each other. Analysis of the wrist segment’s sagittal plane revealed significant differences in angular velocity across various distances (F = 6.69, p < 0.005). Post-hoc comparisons indicated a progressive increase in flexion velocity with increasing distances. Like the elbow joint, all three distances displayed statistically significant differences (p < 0.005) in angular velocity. The wrist joint’s horizontal plane also exhibited significant variations in angular velocity across distances (F = 3.97, p < 0.05). Post-hoc analysis revealed a trend of increasing internal rotation velocity with increasing distances. However, a significant difference in angular velocity was only detected between close and far distances (p < 0.05).
Discussion
Given that the arm holding the racket primarily moves along the sagittal plane during the swing and impact phases [21], the upper limb joints are engaged in flexion/extension and internal/external rotation movements during these stages [22]. Consequently, this study focused on analyzing these specific joint motions.
This study investigated the influence of smash distance on upper limb muscle activation and joint kinematics. Three distinct smash distances (near, intermediate, and far) were employed to evaluate how the upper limb adapts muscular and joint control strategies. Except for the BICLO, all other investigated upper limb muscles (DELTA, DELTL, TRIL, ECR, and FCU) exhibited increased activation levels with increasing smash distance during the stroke. Upper limb joint angle analysis revealed decreased internal rotation angles across all joints (except the shoulder) at the moment of impact with increasing smash distances. Conversely, the angular velocity of all upper limb joints increased with increasing distances.
A recent study [23] reported significant differences between professional players and amateurs in internal and external rotation torques of the shoulder, elbow, and wrist joints during smashes. They observed greater upper limb joint torques in professional players than amateurs, with particularly pronounced increased activation in the DELTA, TRIL, ECR, and FCU muscles.
Additionally, a study found that the activation levels of the shoulder and elbow joint muscles during the acceleration phase of the smash were significantly higher in professional players compared to amateurs [19]. The degree of muscle recruitment during the stroke has been shown to influence badminton flight speed. Ra- masamy et al. [24] reported a significant correlation between badminton flight speed and the angular velocity of the upper limb joints. A faster flight speed results in a longer drop for the shuttlecock at the same initial launch angle. This aligns with the findings of the present study, where muscle activation in the upper limb increased with increasing smash distances. Similarly, Sakurai and Ohtsuki [7] identified muscles as a key factor influencing smash control at varying distances. Their findings support our hypothesis that ECR and FcU are the primary muscles involved in controlling the accuracy of the drop point during smashes [7]. Distal muscles play a crucial role in precision control during badminton strokes, with higher distal muscle activation leading to faster shuttlecock speeds. The relative position of the body and the shuttlecock can influence the falling speed and trajectory. [2] However, when the position and height of the shots are fixed, adjustments to compensate for the previous shot need to be made using the far side of the body. This can lead to situations where the power generated by distal muscles becomes more prominent. This explains why amateur players struggle to hit the desired target consistently, as they lack the ability to coordinate muscle and joint angles effectively. Sakurai and Oht- suki [7] further reported that the FcU exhibits the highest level of muscle activity during the stroke, as it is the primary extensor muscle used to generate force during the swing. Given the established correlation between ball speed and racket head speed, longer distances necessitate greater swing and ball speeds [25]. Higher racket head speed requires greater joint angular velocity, which demands increased muscle activation due to the whipping motion characteristic of the badminton smash [18]. Consequently, as the smash distance increases, the power output of each muscle gradually increases, ultimately leading to a higher overall power output. This enhanced power translates to a faster swing speed, resulting in a longer drop for the shuttlecock.
Previous research by Li et al. [2] demonstrated that variations in the relative position between the body and the shuttlecock can impact the trajectory and speed of the shuttlecock’s descent. In other words, different shoulder joint angles can influence the landing location of the shuttlecock. However, in the present study, no significant differences in shoulder joint angles were observed. This may be attributed to the controlled nature of the experiment, where both the badminton flight path and player position were standardized. Consequently, the shoulder joint angle did not exhibit significant variations during the smash execution. In contrast, the internal rotation angle of the elbow joint and the flexion and internal rotation angles of the wrist joint decreased with increasing smash distances. This suggests that variations in joint angles may influence the angle of contact between the racket and the shuttlecock. A larger internal rotation angle will likely result in a steeper badminton drop trajectory [26]. These findings also suggest that professional athletes can achieve the desired effect by coordinating power generation across multiple joints, which aligns generally with the results reported in the literature [3]. Therefore, as the smash distance decreases, the internal rotation angles of the elbow and wrist are likely to increase.
Analysis of joint angular velocities revealed significant increases in shoulder joint flexion, elbow joint extension, and wrist internal rotation with increasing smash distances. These findings provide compelling evidence that variations in smash distance influence joint angular velocities. Athletes can manipulate racket head speed by increasing joint angular velocity and joint torque [27]. This observation aligns with the study’s hypothesis that varying hitting distances lead to distinct joint angular velocities, which subsequently impact racket head velocity. The flight speed of a badminton shuttlecock significantly attenuates over time due to air resistance [28]. As a result, the shuttlecock travels faster at the beginning of its trajectory, particularly at higher launch positions where the attenuation effect is less pronounced. TO achieve a longer smash distance, a greater initial shuttlecock speed is necessary. Consequently, the angular velocity of each joint must increase proportionally. The wrist joint, located at the distal end of the kinetic chain, plays a crucial role in the whipping action and is essential for shuttlecock control [29]. Professional players exhibit greater wrist involvement compared to amateurs, particularly in terms of precision control, which translates to enhanced control over the shuttlecock’s falling speed and accuracy [30].
The findings of this study offer valuable insights for badminton coaches and athletes to improve their understanding of upper body mechanics during smashes. This knowledge can be directly applied in coaching and training to optimize swing power muscle recruitment based on specific smash requirements. By doing so, athletes can minimize unnecessary energy expenditure and reduce technical errors (turnovers) during gameplay. Furthermore, these results can contribute to advancements in clinical medicine by providing a deeper understanding of the causes and mechanisms underlying upper limb badminton injuries. Research suggests that many badminton players experience shoulder and wrist injuries to varying degrees. During high- intensity competitions, these injuries often stem from postural deviations and insufficient strength during rapid movements [31].
The current study design possesses limitations that warrant consideration. The experiment was conducted in a controlled environment, potentially limiting the generalizability of the findings to real-world game settings experienced by higher-level athletes. Additionally, the enclosed environment might have compromised air circulation, potentially influencing the players’ perception of shuttlecock speed. Furthermore, the non-professional badminton attire may not have accurately replicated the conditions encountered during actual matches. While the participating athletes possessed a background in badminton training, the absence of left-handed players restricted the scope of the study’s observations. To enrich the experimental data, future investigations aim to include amateur, left-handed, and female players. By incorporating these diverse groups and comparing smash mechanics across varying distances, we can broaden the study’s scope and facilitate more comprehensive discussions. However, it is important to acknowledge that the current findings may not directly apply to female, left-handed, or amateur badminton players.
Conclusions
This study revealed that upper limb muscle activation and joint angular velocity increase with increasing smash distances. However, joint internal rotation angles decrease as the distance increases. Based on these findings, we recommend that for close-range smashes, players attempt to internally rotate their wrists to increase the internal rotation angle while maintaining a stable shoulder joint angle at impact. Conversely, for long-range smashes, players should strive to maintain a straighter wrist and arm position to maximize power transfer.