VISUAL ABSTRACT
Introduction
Cardiac autonomic recovery (CAR) reflects the ability of the autonomic nervous system (ANS) to restore cardiac homeostasis [1, 2]. CAR can be improved through lifestyle adaptations, such as healthy eating habits, better sleep patterns, regular physical activity, and sports practice [3, 4]. Its efficiency is an indicator of cardiovascular health, and CAR monitoring can be done via electrocardiogram by assessing resting heart rate variability (HRV), heart rate (HR) behaviour, or the heart rate recovery index (HRRI) after physical exercise [5]. The electrocardiogram, using electrodes, captures the activity of heartbeats related to ventricular contraction and repolarisation and records them in graph form [6].
CAR is of interest to sports and exercise professionals as it allows monitoring of recovery after exercise, which influences the intensity of subsequent training loads. therefore, practical means of monitoring CAR, such as short-range radio telemetry, are necessary for tracking HR and HRV behaviour [5]. High-intensity interval training models utilise a short intervention time, making their use in sports training and physical education attractive [7, 8]. this training model is particularly beneficial for improving maximal oxygen uptake and positively affecting the cardiovascular system in adolescents. In sports, a high-intensity interval exercise model commonly used to improve physical fitness is repeated sprint exercise (RSE) [8], which promotes high discharges of stress substances [8, 9] (e.g., catecholamines), which disrupt the heartbeat.
Particularly in sports of all competition levels, practitioners need to optimise CAR to maintain performance [10, 11]. So, it is essential to understand how to improve CAR in sports practitioners, regardless of age. Especially to understand whether progressive RSE sessions can be conducted on the same day or week of training. Previous analyses indicated that, during and after RSE, adolescent athletes recover more heartbeats than adult athletes [12]. this suggests improved postexercise parasympathetic reactivation, reflecting better CAR. However, heartbeat recoveries alone do not fully reflect CAR, making it necessary to analyse other parameters, such as HRV, for example. However, the effects of RSE on the CAR of adolescent athletes remain limited [12-14]. Like adults, adolescent athletes frequently undergo RSE in sports training, making it interesting to understand CAR after RSE in this population.
The maturation of the CAR shows specific characteristics. there seems to be a gradual decrease in sympathetic activity from infancy to adolescence; whereas parasympathetic activity develops in a cubic shape with an exponential increase and a plateau phase from infancy to middle childhood, followed by a decrease in adolescence [14]. Biological maturation (BM) itself (i.e., the rate of improvement of the biological systems in the human body) [12, 13] varies amongst individuals of similar ages. Exhibiting distinct patterns by sex, girls’ bodies can reach full maturity around the age of 11, while in boys, this occurs later, around the age of 14 [15]. This can affect the rate of maturity of the autonomic cardiac system, indicating the importance of BM influences on cardiovascular factors [14].
Therefore, following the hypothesis that adolescents and adults present significant differences in resting HRV after RSE, the objective of the present study was to analyse the effect of RSE on CAR, assessed by the HR recovery index and the time and frequency domains of HRV in male adolescents and adults.
Material and methods
Study design and participants
This experimental, non-randomised trial with a final sample of 38 physically active male individuals (22 adolescents [age, year: 12.7 ± 1.2] and 16 adults [age, years: 22.6 ± 2.2]) who practised intermittent sports (Brazilian jiu-jitsu, karate, football, volleyball, and basketball). Of the participants, 30% were national-level competitors, 60% were regional-level competitors, and 10% were recreational-level practitioners. Participants were recruited randomly from gyms and sports clubs, and the following inclusion criteria were considered: (i) Being involved in a sport for at least one year prior to the research; (ii) Having a minimum training frequency of two sessions per week (60 min/session) in the six months prior to the research; (iii) Aiming for a high level of physical activity (equal to or greater than 3.000 METs per week). The exclusion criteria comprised: (i) Taking any medication that could interfere with CAR (e.g., antidepressants); (ii) Using a supplement that could act as a stimulant (e.g., caffeine and taurine) or that interacts with cardiac recovery (e.g., dilating vessels); (iii) Having a clinically diagnosed cardiovascular disease (e.g., high blood pressure and atrial fibrillation); and (iv) Having suffered any musculoskeletal injury in the six months prior to the research. Participants were instructed not to engage in intense physical activities and to maintain their dietary patterns during the course of this study.
Sample calculation a priori
Sample size defined a priori based on a pilot study of 20 subjects (10 adults and 10 adolescent males). this sample was subjected to an RSE protocol (three rounds of 6 sprints interspersed with 5 minutes of passive rest) [12]. the protocol was performed on an official athletics track (temperature 27.0 ± 1.4°C, air humidity 65.7 ± 3.6%, thermal sensation 30.8 ± 3.9, wind temperature 25.9 ± 1.5, and wind speed 0.2 ± 0.1 m/s). We analysed the HRRI (60 seconds and 300 seconds) during (after rounds 1 and 2 of RSE) and immediately after RSE (end of round 3). We also evaluated HRV measurements (the mean square root of successive differences in intervals between heartbeats (RMSSD) and low frequency/high frequency ratio (LF/HF). We consider the effect of time (Heartbeat index: 60 seconds, 120 seconds, 180 seconds, 240 seconds, and 300 seconds and HRV: pre, 20 minutes, 2 hours and 24 hours post-RSE) and condition (adolescents vs adults). thus, based on the rfp values of 0.35 found in the analyses, we performed a sample size calculation using open-source software G*Power (version 3.1, Düsseldorf, Germany) [16], Considering a standard a of 0.05 and a β of 0.90. therefore, a minimum sample size of 16 individuals was indicated for the adult group and 16 for the adolescent group (Sample power: 0.93 and Critical F: 4.6). To prevent possible sample losses, we sought to recruit 40% more than the sample size recommended by the calculation. During recruitment, six adult volunteers were excluded due to caffeine supplementation and three adolescent volunteers were excluded for not meeting the minimum physical activity level required by the present study (Figure 1).
Procedures
Participants were first assessed for their anthropometric, hemodynamic, fitness, and sleep status before engaging in the RSE protocol. the following day, participants arrived at the data collection site at 7 a.m. (they sat and rested for 10 minutes), so we performed HRV analysis at rest (by short-range radio telemetry in a climate-controlled room at 24°C). Next, participants were directed to an athletics track, where they performed a brief warm-up (5 minutes) involving jumping and short walks. After heating, the RSE started (Figure 2). the analyses took place from Monday to thursday, beginning at 7 a.m. and ending between 7:40 and 8 a.m. (on the day of sample characterisation and the RSE day). the procedures were carried out by a research team composed of an anthropometrist for the collection of anthropometric data and body composition analysis, a nurse for the collection of hemodynamic data, two nutrition professionals for the evaluation of nutritional patterns, three physical education professionals for the RSE interventions and analysis of physical activity levels, and two first-aid workers in case of emergencies during the interventions
Characterisation of the sample
Anthropometry
With participants barefoot and wearing light clothing, body mass was measured using a Filizola® digital scale with a capacity of 200 kg and a variation of 0.10 kg (Sao Paulo, Brazil). Height was determined using the Sanny® stadiometer (accuracy of 0.1 mm, Sao Paulo, Brazil). All measurements were made by a single assessor using the International Society for the Advancement of Kinanthropometry (ISAK) protocols. For the technical error of intra-observer anthropometric measurements, we used the acceptable magnitude [17] of < 1.0%. We highlight that to trace the maturation profile of pediatric participants, the height of their parents was also measured. From the height and body weight data, we calculated the body surface area (BSA) using the formula [18]:
BSA = [body mass (kg)0.5378] x [height (cm)0.3964] x 0.024265.
We determined body mass index (BMI) by dividing body weight in kilograms by height in meters squared (BMI = body mass (kg)/height (m2).
Hemodynamic variables
Blood pressure was measured by a single evaluator after participants rested for 10 minutes, sitting in a comfortable chair with a backrest, and knees positioned at 90°. The evaluator used a manual sphygmomanometer (BIC®, Sao Paulo, Brazil) with a stethoscope (Premium®, Sao Paulo, Brazil) to register systolic and diastolic pressure. Resting HR and blood oxygen saturation were checked with the aid of a portable oximeter (G-Tech®, Sao Paulo, Brazil) positioned on the index finger of the left hand. The body’s blood volume was estimated based on the following mathematical model [19]:
Body blood volume (ml) = [(0.006012 x height (inches)3] + [14.6 x body mass (pounds)] +604
Physical activity levels
The participant’s level of physical activity was analysed using the web version of the International Physical Activity Questionnaire (web-IPAQ, http://www.webipaq.com.br/). The questionnaire is divided into sections: (i) Physical activity at work (for adults), (ii) Physical activity as a means of transportation and Domestic physical activity carried out at home, and iii) Leisure and sports physical activities. The web-IPAQ automatically calculates the metabolic equivalents (METs) referring to the energy expenditure per physical activity [20] of a usual week, providing a score for each session and the total score. Using total METs, we classified the participants’ level of physical activity as low (> 600 to < 1.000 METs), moderate (> 1.000 to < 3.000 METs), and high (equal to or above 3.000 METs). The web-IPAQ was answered based on usual activities over the last three months, with the presence of at least one of the tutors (for adolescents) and a researcher who helped the participants interpret the questions (adolescents and adults).
Body composition
Levels of lean mass, fat-free mass, and fat mass were analysed by dual-energy X-ray absorptiometry (DXA, LUNAR®/GE PRODIGY - LNR 41,990, Washington, DC, United States. enCORE Software, GE Healthcare®, version 15.0, Madison, WI, USA) and algorithms appropriate for the paediatric population [21]. The following DXA configuration was used for the evaluations: whole body evaluation, voltage (kV): 76.0, current (mA): 0.150, and radiation dose (pGy): 0.4 (very low, no risk to the health).
Sleep efficiency
Sleep efficiency was recorded according to the protocol proposed by Reed and Sacco [22] and used as a control variable. The protocol involves using information from a sleep diary to calculate sleep efficiency. Participants recorded in the sleep diary the time they went to bed intending to sleep, the estimated time they took to fall asleep (latency), the times they woke up and went back to sleep after getting out of bed, and the wake-up time. Sleep efficiency was considered ideal when the values reached were equal to or greater than 85% [22]. During the present study, all participants showed sleep efficiency above 85%. In the present study, sleep efficiency assessments conducted 24 hours before and 24 hours after the RSE indicated high reliability [CCC: 0.92 (95% CI: 0.89; 0.98)]. The mathematical model for analysing sleep efficiency consisted of:
Sleep efficiency = [TTS (min) / (SL + TTS (min) + AWMT (min) + TBTBFW (min)] x 100,
where TTS - total time sleeping, SL - sleep latency, AWMT - awake mid-time, TBTBFW - time before fully waking
Nutritional pattern
Food recall questionnaires (last 24 hours - R-24h) were used: the first was administered 24 hours before the intervention [recall of the weekend (Sunday), a day of atypical eating], the second on the day of the intervention, and the third on the day after the intervention (typical eating days) [23]. The R-24h questionnaire consisted of recording the home-cooked portions [small, medium, or large] of all meals eaten the previous day as a reminder [23, 24]. Adolescent participants were assisted by their guardians in filling out the R-24h. The R-24h was analysed by nutrition professionals who confirmed that the dietary pattern did not change during the present study. In this regard, the reliability of the analysed questionnaires were good [CCC: 0.85 (95% CI: 0.79; 0.88)].
Biological maturation profile
The BM profile was determined based on the comparison of adolescents (subjects in the process of maturation) and adults (mature subjects) and based on the estimation of the percentage of final height as an adult and peak height velocity (PHV). In this way, the percentage of final height was acquired from the mathematical model proposed by Khamis and Roche [25] or children and adolescents of both sexes aged between 4 and 17.5 years:
Final height (inches) = -2.87645 + height (inches) (1.11342) + body mass (pounds) (-0.013184) + average height of parents (inches) (0.32748)
Subsequently, the result of the Khamis and Roche [25] equation was considered as 100% of the final height. We then multiply the current height by 100 and divide it by 100% of the adolescent’s predicted final height, thus determining the current percentage of the final height. The PHV stage was determined by the mathematical model proposed by Moore et al. [26] for male subjects aged between 8 and 18 years [the equation explains 89.6% of the variation in PHV in males (r2 = 0.896, standard error = 0.542)]:
PHV = -7.999994 + [0.0036124 x (age (years) x height (cm))],
where PHV - peak height velocity
Based on the results of the mathematical model, the PHV can be classified into [26]: late (< -1), circum (between -1 and 1), and early (> 1).
Intervention with RSE protocol
The RSE consisted of three rounds of six 35-meter sprints interspersed with 10 seconds of interval and divided by a 5-minute passive rest sitting in a chair with a backrest with their knees flexed at 90° (Figure 2). Two timekeepers monitored the intervals between sprints, and another timekeeper counted the resting time between rounds with digital stopwatches (Monalisa®, Sao Paulo, Brazil). Participants received verbal encouragement, and the RSE was performed on an official track field (temperature 27.3 ± 1.2°C, air humidity 66.3 ± 4.0%, thermal sensation 29.5 ± 3.5, wind temperature 26.0 ± 1.3, and wind speed 0.2 ± 0.1 m/s).
On the intervention day, participants arrived at the data collection site at 7 a.m. to perform the HRV analysis at rest. Then, they were directed to the track field and briefly warmed up for 5 minutes with jumps and short walks before the RSE started. HR was monitored throughout the whole RSE execution and recovery using short-range radio telemetry. Participant’s HRV was assessed during the RSE and again at 20 minutes, 2 hours and 24 hours after the RSE (Figure 2). All participants consumed their last meal at least 2 hours before the procedures began and abstained from intensive exercise in the 24 hours prior to the aforementioned procedures.
Rating of perceived exertion
The rating of perceived exertion (RPE) was monitored using the scale proposed by Borg [27] (validity: r2 = 0.81. The scale was validated for the Brazilian population [28]), and the HR was monitored by radio telemetry during the RSE. A monochromatic visual scale with numerical values between 6 and 20, where 6 indicates absolute rest and 20 maximum effort, was used. Post-intervention (RSE), all groups reported an RPE in the high-intensity range (adolescents: 17.6 ± 1.5, adults: 17.1 ± 1.8). The participants were familiarised with the RPE the day before the intervention and were asked to indicate the set of all RSE rounds. For familiarisation, we presented the RPE to the participants and explained that point 6 on the scale represents total rest and point 20, total exhaustion. Subsequently, they were instructed to perform a brief three-metre walk in a straight line and were asked, in relation to rest (point 6 on the scale), about the effort of the brief walk. Then, they were asked to perform a brief three-metre run and return to the starting point and were again asked about the effort in relation to rest (point 6 on the scale).
Heart rate recovery index
For the HRR assessment, participants were seated on a chair with a backrest and knees at 90° in a tented environment. We used short-range radio telemetry with the aid of a Polar® HR monitor (Model H10, Kempele, Finland) attached to the subject’s chest with a corresponding Polar® strap (validity in relation to echocardiogram: r2 = 0.90, ICC: 0.93 [29]). Then, according to the recommendations of previous studies [5, 30, 31], we subtracted the HRR of the recovery moments (60 seconds, 120 seconds, 180 seconds, 240 seconds, and 300 seconds) from the peak HR recorded during the RSE. In this manner, we acquired the HRRI at each recovery phase: (i) fast recovery (at the end of 60 seconds of rest), (ii) intermediate recovery (at the end of 120 seconds, 180 seconds, and 240 seconds of rest), and (iii) slow recovery (at the end of 300 seconds of rest) [5].
Heart rate variability
To assess the HRV, participants were sitting for 10 minutes with knees at 90°. Then, the short-range radio telemetry equipment was activated via smartphone through the Elite HRV® application (Gloucester, Massachusetts, USA), and the HRV assessment began to be recorded. Participants were asked not to speak, sleep, or make sudden movements. The HRV acquisition process lasted 10 minutes, and the assessments were saved in text file format in the Elite HRV® application and imported into the Kubios HRV® software (Version 3.3.1, Kuopio, Finland). In Kubios, low-level artefact correction was applied, and the sample length was set to 5 minutes (short-term) for the HRV process. Additionally, we considered the percentage of corrected beats to be 2%. In this sense, we evaluated as a time-domain measure the RMSSD, which expresses a representation of vagal activity [32]. For the frequency domain, we use the LF, which reflects the activity of the sympathetic system, the HF, which represents parasympathetic modulation, and the ratio between LF and HF (LF/HF), which is used to suggest levels of sympathetic-vagal modulation [32]. All assessments were carried out in the laboratory (temperature 24°C).
Statistical analysis
Data analysis was processed by a blind external collaborator. Descriptive data was analysed based on the recommendations of Mishra et al. [33]. The normality of the data of the independent variables (considering the time factor) was verified by the Shapiro-Wilk test, Z score of Asymmetry and Kurtosis (-1.96 to 1.96), and by QQ-line plotting. The percentage of the coefficient of variation of the variables (CV%) of sample characteristics was calculated using the formula:
CV% = (standard deviation/mean) x 100.
Levene’s test verified the assumption of homogeneity of variances for each independent variable (age group and BM). Furthermore, the sphericity of the data was verified using the Mauchly test. For comparative analyses of HRR indices, the assumption of data normality was not denied. Therefore, we used the General Linear Model (GLM) [34, 35]. In the GLM analyses, we considered the factors time (60 seconds vs. 120 seconds vs. 180 seconds vs. 240 seconds vs. 300 seconds), age (adolescents vs. adults), and BM (pre-PHV vs. circum-PHV vs. adults).
For comparisons of sleep efficiency and HRV variables, the data showed a non-parametric distribution; therefore, we used the Kruskal-Wallis test to compare the aforementioned variables. Therefore, the HRV and sleep efficiency variables were expressed as median and interquartile ranges. For sleep and HRV analyses, we also considered the time factor (sleep: 24 hours before, day of RSE, and 24 hours after RSE; HRV: pre, 20 minutes, 2 hours, and 24 hours after RSE), and condition (age group and BM).
Point differences in all analyses were verified by Bonferroni post hoc. Thus, the effect size between the differences was verified by partial eta-squared (rfp) considering the magnitude [36]: small (< 0.01), medium (between 0.02 and 0.06), and large (> 0.14). For all analyses, a significance level of p < 0.05 was considered. All analyses were performed using the open-source software Jamovi® (Version 2.3.18, Sydney, Australia). All figures analyses were performed in GraphPad Prism® software (Version 8.01 (244), California, USA).
Results
Three adolescent participants were not included due to the criteria of the required level of physical activity, and six adults were excluded because they were taking caffeine supplements. The final sample of the present study was composed of 38 physically active individuals (22 adolescent and 16 male adults) from Brazilian jiu-jitsu, karate, football, volleyball, and basketball; 30% of whom were national-level competitors, 60% regional-level competitors, and 10% recreational-level practitioners (Figure 1). Table 1 shows the sample characteristics in relation to BM and anthropometric, healthy, and cardiovascular parameters.
Table 1
Sample characterization
In the present study, there were no significant differences (adolescents and adults: p > 0.05, rfp < 0.02) between the medians for the percentage of sleep efficiency in the moments 24 hours before RSE (adolescents: median [interquartile range] = 97.1 [4.6]. Adults: median [interquartile range] = 95.2 [6.9]), on the day of RSE (adolescents: median [interquartile range] = 97.6 [4.3]. Adults: median [interquartile range] = 94.6 [11.0]), and 24 hours after RSE (adolescents: median [interquartile range] = 95.7 [4.9]. Adults: median [interquartile range] = 94.8 [9.7]). In relation to adults, the descrip tive analysis of the HRR indicated that adolescents achieved a more efficient parasympathetic reactivation than adults (Figure 3A), especially those in the pre-PHV stage of BM (Figure 3B).
Figure 3
Descriptive analysis of the pattern of heart rate recovery after repeated sprint exercise rounds: A – adult and adolescent sample and B – sample of adults and stratification of adolescents according to the stage of biological maturation
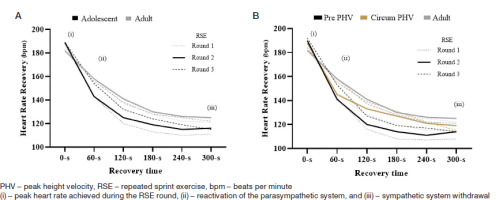
When considering the number of heartbeats recovered in relation to the peak HR achieved during the RSE rounds, there was an effect of time (60 seconds to 300 seconds) and condition (adolescents vs. adults) favouring adolescents, who showed better HRR rates in all RSE rounds (Table 2). Sub-analyses showed that, in general, adolescent participants in the pre-PHV BM stage had a higher HRR rate compared to their circum-PHV peers and adults (table 2).
For HRV, we identified an effect of time (pre, 20 minutes, 2 hours, and 24 hours post-RSE) for the RMSSD (rfp = 0.280) and the LF/HF ratio (rfp = 0.129, Figure 4). An effect of condition (adolescent vs adult) was indicated for the LF/HF ratio (t]2p = 0.045, Figure 4). the sub-analyses showed that regardless of the maturation stage (condition: pre-PHV vs. circum-PHV vs. adult), there was an effect of time (rfp: 0.210) and time-condition interaction (r|2p = 0.172) for RMSSD (Figure 5). For LF, there was an interaction effect between time and condition (rfp = 0.128), and for the LF/HF ratio, there was an effect of time (rfp = 0.092) and condition (rfp = 0.052, Figure 5).
Figure 4
Comparisons of heart rate variability variables considering adolescent and adult groups: A - analysis related to RMSSD, B - analysis related to LF, C - analysis related to HF. Analysis related to LF/HF
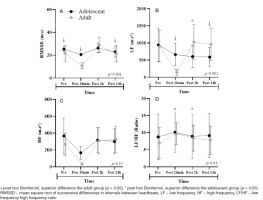
Figure 5
Comparisons of heart rate variability variables among the pre-PHV, circum-PHV, and adult groups: A - analysis related to RMSSD, B - analysis related to LF, C - analysis related to HF. Analysis related to LF/HF
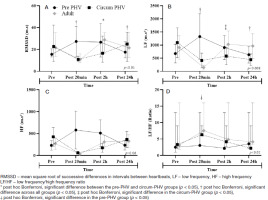
In addition, adolescent participants reported higher RMSSD values than adults for the pre-, 20-minute, and 24-hour moments after RSE (Figure 4A). For LF, adolescent participants showed higher values than adults for the moments 20 minutes after and 24 hours after RSE, while adults showed higher values than adolescent participants only at the moment 2 hours after RSE (Figure 4B). For HF, there were no significant differences between the groups (Figure 4C). For the LF/HF ratio, adults showed higher values than adolescent participants at moments 20 minutes and 2 hours after RSE (Figure 4D).
The sub-analyses considering the stages of BM showed that for the RMSSD, the pre-PHV group showed higher values than the circum-PHV and adult groups at the moment 20 minutes after the RSE. the adult group was superior to the pre-PHV and circum-PHV groups at 2 hours after the RSE. However, 24 hours after the RSE, the circum-PHV group showed higher RMSSD values than the other groups (Figure 5A). For LF, the pre-PHV group showed higher values than the other groups at the moment 20 minutes after the RSE. Two hours after the RSE, the pre-PHV and adult groups showed higher values than the circum-PHV group, and 24 hours after the RSE, the adult group showed higher values than the other groups (Figure 5B). For HF, there were no significant differences between the groups (Figure 5C). For the LF/HF ratio, the circum-PHV and adult groups showed higher values than the pre-PHV group (Figure 5D).
Discussion
In this study, we objectively analysed the effect of RSE on CAR, assessed by the HRRI and time and frequency domains of HRV in male adolescents and adults, and we confirmed our hypothesis that the CAR was different between adolescents and adults after RSE. Further investigation showed that this difference was based on different HRR and HRV responses. Further more, the alterations in the CAR may be related to BM in a manner that individuals in the pre-PHV stage of BM may have advantages compared to their circum-PHV peers and adults.
Cardiac autonomic recovery and repeated sprint exercise
After the RSE, the CAR showed an alteration towards greater sympathetic activity and less parasympathetic activity, which took a long time to stabilise [37]. This phenomenon reflects a norepinephrine discharge that occurs during sub-maximal efforts, and the elevated levels of local metabolites, such as hydrogen (H+) and inorganic phosphate (Pi), during the recovery period [38, 39]. Indeed, the physiological disturbances of RSE downregulated the parasympathetic activity for at least 2 hours after a stimulus [40, 41]. This may explain why the LF component of HRV increased and the RMSSD component reduced after RSE, as LF and RMSSD are indirect markers of sympathetic and parasympathetic activity, respectively (Figures 4 and 5) [42]. Similar results have been previously reported after RSE protocols (4 x Wingate 30 seconds interspersed with 4 minutes of light cycling at 4W) [37].
Previously, it was observed that in cycle ergometric exercises, CAR recovery may be stabilised a few hours after exercise [43]. However, the present study identified that after RSE, HRV components were fully recovered 24 hours later (Figure 4). This finding aligns with suggestions from previous studies indicating complete CAR recovery 24 hours after RSE [44]. Apparently, exercise modes involving more muscle groups may require more time to stabilise the CAR (e.g., running versus cycling) [45]. In this context, supra-maximal exercises performed through running require greater muscle activity and engagement compared to exercises performed on cycle ergometers or at light to moderate intensities [46]. This may explain why the present study found CAR to be significantly restored 24 hours after RSE.
Therefore, adequate recovery after anaerobic activities such as RSE can apparently influence the performance of adolescent and adult athletes and may be decisive for the sporting success of athletes in intermittent sports [47, 48]. Previously, it was observed that football players who have better CAR, have a lower percentage of sprint decrease, optimising performance during matches [49]. Thus, CAR appears to be closely related to improving the athlete’s ability to maintain good performance levels during RSE, since a better CAR allows maintenance for subsequent efforts.
We highlight that, in the present study, the participants showed a similar level of physical activity (high, above 3.000 METs) and practised various intermittent sports (combat or court sports). However, previous studies have indicated that elite athletes from different modalities (endurance, strength-sprinter, and ball-game players) did not show significant differences in CAR parameters after exercise [50]. Apparently, for CAR, the level of physical activity is more important than the type of sport practised [5]. This suggests that the results of the present study can be generalised to individuals from other sporting modalities that show a similar level of physical activity to the sample in the present study.
Cardiac autonomic recovery in adolescents and adults
Adolescents and adults present unique ANS in relation to cardiac function. During childhood, the excitability of the sympathetic and parasympathetic systems is the greatest, decreasing throughout the transition between childhood and adolescence [14]. A decrease in baroreflex sensitivity, that accompanies aging, may contribute to this since the baroreflex is an indirect marker of sympathovagal interaction [51, 52]. During adulthood, there is a plateau in ANS activity, which subsequently decreases as the individual ages [53]. Therefore, when we compared the CAR of adolescents and adults, we were able to find a significant difference between recovery and cardiac modulation. Our results indicate that there is a faster reactivation of the parasympathetic nervous system in adolescents, as interpreted by HRR and HRV (see Figures 3-5), contributing to a more efficient CAR, as interpreted by a restoration of CAR to baseline levels, which corroborates other findings in the literature [5, 14].
In the present study, when we analysed the percentage of resting HR, we identified that at the end of the 300-second rest intervals between rounds, and at the end of RSE, the adolescent’s HRR values were 66.6% above the resting (baseline) HR (Figure 3A). Meanwhile, adults showed an HRR of 57% above the resting HR (Figure 3A). Additionally, the present study suggests that sympathetic-vagal modulation and parasympathetic re-stabilisation was better in adolescents (Table 2, Figures 4 and 5). Both findings can be justified because the CAR kinetics observed in adolescents are related to a lower absolute work rate of the less developed anaerobic function [54]. Blood lactate concentrations, muscle acidosis, and average power are lower in adolescents compared to adults [46, 54]. Furthermore, the muscle structure of adolescents is smaller than that of adults, which can affect glycogen stores during sprint exercises [46].
Table 2
Comparisons of the heart rate recovery index considering the adolescent and adult groups, and the sub-analyses considering the stages of biological maturation
In another study, it was observed that in adults, following RSE, the HRRI was dependent on anaerobic contributions and exercise intensities, while HR variability (RMSSD30s) was impaired, resulting in slower parasympathetic recovery, especially in the first 10 minutes after RSE [40]. Additionally, in a separate research study, parasympathetic activity was found to be impaired in young soccer athletes compared to resting parasympathetic activity analysed by HRV (InRMSSD) at 1 and 2 hours post-RSE [41]. These findings suggest that RSE suppresses parasympathetic activity in both young and adult populations, supporting the findings of the present study.
Meanwhile, in the present study of the adolescent group, the parasympathetic activity was less impaired compared to the adult group (Table 2, Figures 4 and 5). One possible explanation for this is that in resting states, younger individuals have lower circulating levels of norepinephrine, an excitatory neurotransmitter for the sympathetic nervous system, compared to older subjects [55]. This neurotransmitter triggers the a-adrenergic-II agonist response, which antagonises the α-adrenergic-I receptor. A mechanism that results in vasoconstriction and leads to increased systemic resistance and elevation of blood pressure and HR [5658], all of which are sympathetic responses that [47-49] negatively affect the CAR.
Cardiac autonomic recovery and biological maturation
Adolescents show distinct CAR patterns according to their BM stages [14], and more immature subjects seem to benefit [12]. A possible explanation could be the high mitochondrial density [46, 59] and the highest percentage of aerobic enzymes present in immature subjects (e.g., carnitine palmitoyltransferase and 2-oxoglutarate dehydrogenase) [60]. Another influencing factor is the smaller overall body size, which favours the cardiorespiratory system due to the shorter vascular connections between the cardiac and respiratory systems, and better gas exchange between tissues that optimise CAR [59, 61, 62]. In the present study, when analysing the results of the sub-analyses considering stages of BM to assess HRR patterns, it was observed that during the 300-second intervals between rounds and at the end of RSE, pre-PHV subjects showed a 70.5% higher HRR compared to resting HR, while circum-PHV subjects showed approximately 61.6% above resting HR (Figure 3B), indicating a difference in HRR of 8.9%. This suggests that among adolescent participants, the BM stage influences HRR.
Previously, it has been pointed out that BM analysed by age groups also showed different patterns in relation to CAR. To this end, data were gathered from five large studies that obtained data from electrocardiogram exams on the behaviour of CAR and analysis of parasympathetic and sympathetic activities in subjects from 0.5 to 20 years of age [14]. It was found that there is an increase in parasympathetic activity after birth, a plateau in childhood, and a decrease in late adolescence, while sympathetic activity shows a decrease during all ages [14]. This corroborates the present study, where we identified that the reactivation of the parasympathetic nervous system and the withdrawal of the sympathetic nervous system are more efficient in more immature adolescents (in the pre-PHV BM stage, Figure 3B, Table 2). For HRV, we also identified significant differences between BM stages for time (RMSSD) and frequency (LF) domain variables (Figures 5A and 5B).
Buchheit et al. [63] analysed male soccer athletes, aged between 12 and 18 years, and did not find a distinct effect of maximal running exercise on HRV parameters at different stages of BM analysed by PHV estimation, which contrasts with the findings of the present study. However, it was observed that pre-PHV subjects demonstrated a tendency to present a better CAR than their circum- and post-PHV counterparts. A possible explanation for the discrepancy in results between the aforementioned study and the present research is that the authors employed an incremental exercise model until exhaustion, which may not have been able to induce distinct disturbances in the CAR of subjects at different stages of BM.
The incremental exercises used by the authors started at a speed of 8 km/h, increasing by 1 km/h per minute. This configuration allows the autonomic system to gradually adapt to the training load so that the cardiovascular system can stabilise and exhaustion occurs in the neuromuscular system. In other words, the subjects may not have reached a high level of autonomic cardiac fatigue. This is different from the RSE protocol used in the present study, which consisted of supramaximal stimuli that led the participants to high levels of cardiovascular stress (according to the HR behaviour during the RSE and the HRV results of the present study, especially 20 minutes after the RSE). In this sense, catecholamine surges and cardiovascular work tend to be more intense in the RSE, which may promote greater disturbances in CAR after exercise.
Another explanation for discrepancies between studies is the chronological age of the sample. The average age of participants in the present study was 12.7 years, with a coefficient of variation of only 9.4% (low). It is known that the PHV estimate loses precision when moving away from the PHV event. In boys, PHV tends to occur between 11 and 14 years of age [64, 65]. It is likely that due to the broad age range of the Buchheit et al. study sample (12 to 18 years), the accuracy of the PHV estimate was compromised.
Practical applicability
Coaches and professionals in the area of physical education and sports must be aware of the differential patterns of CAR in adolescents and adults who practice sports, even after short (but intense) sessions of exercise, training, and/or physical tests. Consecutive training sessions without adequately respecting CAR may lead to an unfavourable performance response (e.g., non-functional overreaching and overtraining). The CAR can be monitored between exercise intervals during training through indices based on the HR, and after the training sessions (5 minutes, 10 minutes, 15 minutes, 20 minutes, 1 hour, 2 hours, 6 hours, or 24 hours) through HRV at rest.
Considering that the present study suggests that as long as BM is respected, it is safe to use high-intensity interval exercise models in adolescents without posing risks to CAR. thus, BM can be monitored using user-friendly mathematical models that provide reliable estimates based on easily obtainable anthropometric variables [66, 67]. It is worth noting that previous studies have indicated that high-intensity interval exercise interventions can be useful for promoting cardiovascular health in schoolchildren, reducing body fat, and improving aerobic performance [68]. thus, physical education teachers are encouraged to implement high-intensity interval exercises in physical education classes [69]. they should take into account the sex and individualisation of the training load for an optimised prescription [70]. In this regard, high-intensity interval exercise is a viable approach to improve cardiorespiratory fitness [71], potentially generating beneficial changes in CAR. Finally, we recommend that when conducting interventions with RSE, the involved muscles, especially those of the lower limbs, should also be strengthened [72-74], as this may reduce neuromuscular fatigue and positively influence the maintenance of CAR.
Limitations and suggestions
Despite the relevant results, the present study has the following limitations: (i) Not having analysed other components of the CAR (i.e., blood pressure variability, recovery blood pressure, baroreflex, and metaboreflex). The analysis of more components could provide a more concise answer regarding the effect of RSE and BM in relation to CAR. (ii) We did not analyse a group of middle-aged subjects; this could provide valuable insights regarding the ageing process and CAR behaviour after RSE. (iii) We did not analyse female subjects, making it impossible to extrapolate the data to this population. We suggest that future studies try to overcome these limitations, as well as encourage the production of new studies considering the effect of BM on CAR, especially using exercise models that differ from the one used in the present study.
Conclusions
It was concluded that 20 minutes after RSE, parasympathetic activity in adolescents and adults is significantly impaired, with adolescents being less affected compared to adults, especially those in the pre-PHV BM stage. Apparently, CAR is dependent on the BM stage, suggesting that it is necessary to take BM into consideration when prescribing RSE. It is also concluded that, in adolescents and adults, at least two hours after RSE, parasympathetic activity approaches baseline values, being stable again 24 hours after RSE.